تصنيع الشعاع الكهربائي يشير إلى مجموعة متنوعة من عمليات التصنيع التي تستخدم مصادر الطاقة المركزة مثل أشعة الليزر أو الحزم الإلكترونية أو أقواس البلازما لصهر المواد أو صهرها أو تبخيرها بشكل انتقائي. ومن خلال التحكم الدقيق في مصدر الطاقة، تسمح هذه العمليات بتصنيع دقيق للغاية وقابل للتكرار بأقل قدر من التشويه الحراري. يتيح التصنيع بالحزم الكهربائية إنتاج أشكال هندسية معقدة وميزات دقيقة لا يمكن تحقيقها باستخدام تقنيات التصنيع الطرحي التقليدية.
كيف تعمل عمليات تصنيع الشعاع الكهربائي؟
يعتمد تصنيع الحزمة الكهربائية على مبادئ كثافة الطاقة ونقل الحرارة. من خلال تركيز الطاقة في حزمة ضيقة للغاية، يمكن تحقيق كثافة طاقة عالية جدًا. عندما تتفاعل هذه الطاقة المركزة مع المادة، لا ينصهر أو يتبخر سوى حجم صغير فقط. وتتبدد الحرارة بسرعة من حجم التفاعل الصغير هذا إلى المادة المحيطة التي تظل أكثر برودة. وهذا يسمح بصهر المادة أو صهرها أو تبخيرها بشكل دقيق وموضعي للغاية.
هناك العديد من الأنواع الشائعة لتصنيع الشعاع الكهربائي:
تصنيع أشعة الليزر
- يستخدم شعاع ليزر عالي التركيز كمصدر للطاقة
- يمكن تركيز أشعة الليزر حتى 0.01 مم أو أقل
- يسمح بدقة عالية جدًا وتقليل التشوه الحراري إلى أدنى حد
- تُستخدم في اللحام، والقطع، والكسوة، والتصنيع المضاف، وما إلى ذلك.
تصنيع الحزمة الإلكترونية
- يستخدم حزمة مركزة من الإلكترونات كمصدر للطاقة
- يمكن تركيز حزم الإلكترونات حتى 0.1 مم
- يسمح بالعمل في بيئة مفرغة من الهواء
- تُستخدم في اللحام، والتصنيع المضاف، وما إلى ذلك.
تصنيع قوس البلازما
- تستخدم نفاثة البلازما كمصدر للطاقة
- توفر أقواس البلازما كثافة طاقة عالية
- يسمح بلحام المعادن بثقب المفتاح
- يستخدم في اللحام والقطع عالي الاختراق
في جميع الحالات، يتم تحريك قطعة العمل و/أو مصدر الشعاع عن طريق التحكم الرقمي باستخدام الحاسب الآلي لتتبع الهندسة المطلوبة. تتيح أدوات التحكم بالكمبيوتر والأتمتة أن تكون العملية قابلة للتكرار والاتساق بدرجة كبيرة.
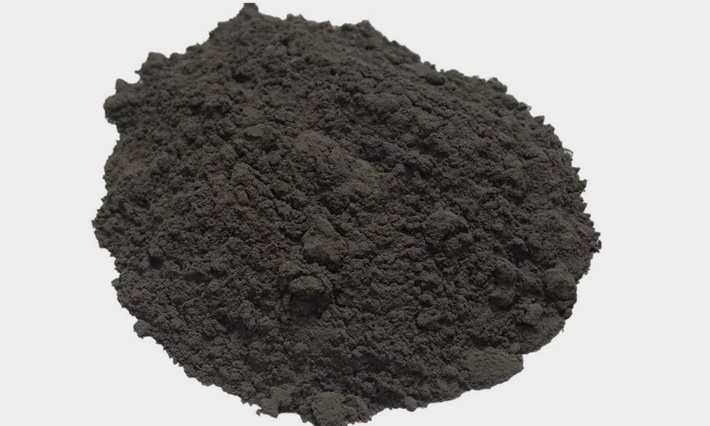
ما هي فوائد تصنيع الشعاع الكهربائي؟
بالمقارنة مع طرق التصنيع التقليدية، يوفر التصنيع بالأشعة الكهربائية العديد من المزايا:
- دقة متناهية –؛ وبفضل حزم الطاقة عالية التركيز، يمكن الحصول على أحجام وتفاوتات تصل إلى 0.01 مم. وهذا يتيح المكونات والإلكترونيات متناهية الصغر.
- الحد الأدنى من التشوه الحراري –؛ يتم صهر كمية صغيرة فقط من المواد في المرة الواحدة. وهذا يسمح بالتبريد السريع ويقلل من التأثيرات الحرارية الضارة. تُظهر الأجزاء التواءً وتشويهًا أقل.
- لا توجد أدوات مطلوبة –؛ يعمل شعاع الطاقة بشكل أساسي كأداة، مما يلغي الحاجة إلى الأدوات المادية. يتيح ذلك إمكانية وضع النماذج الأولية السريعة وتكرار التصميم.
- قابلة للتكرار بشكل كبير –؛ تسمح العوارض التي يتم التحكم فيها باستخدام الحاسب الآلي بمعالجة متناسقة ومتكررة للغاية ومناسبة للإنتاج بكميات كبيرة.
- لا توجد قوى أو ضغوط –؛ نظرًا لعدم وجود تلامس مادي، يمكن عمل الهياكل الحساسة دون ضرر. كما يمكن أيضًا توفير ميزات وهندسة داخلية.
- خصائص معدنية ممتازة –؛ يمكن لمعدلات التبريد السريع أن تخلق هياكل دقيقة الحبيبات وخصائص مواد فريدة من نوعها.
- توافق واسع للمواد –؛ يمكن معالجة المعادن والبوليمرات والمواد المركبة والسيراميك بنجاح مع اختيار المعلمات المناسبة.
وتتيح هذه المزايا استخدام تصنيع الحزمة الكهربائية في مجموعة واسعة من التطبيقات التي لا يمكن إنتاجها بسهولة بوسائل أخرى. وتستمر العمليات في التحسن من حيث الدقة والتكرار والفعالية من حيث التكلفة.
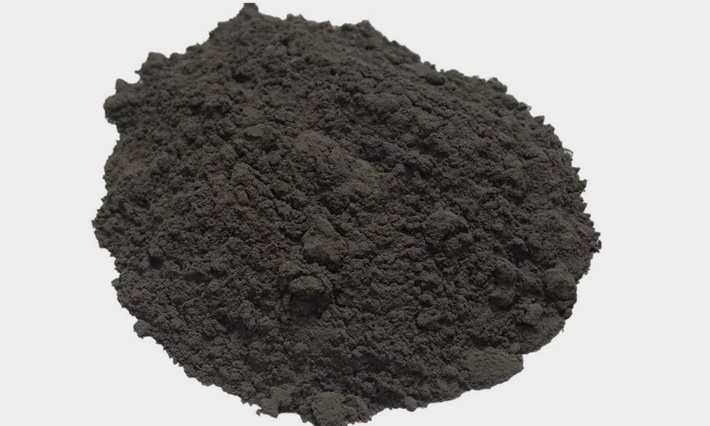
ما هي المواد التي يمكن معالجتها؟
تتوافق العديد من المواد المختلفة مع تصنيع الأشعة الكهربائية:
المعادن –؛ يمكن معالجة جميع المعادن الشائعة بما في ذلك الفولاذ والألومنيوم والتيتانيوم وسبائك النيكل وغيرها. قد تتطلب السبائك والخلائط المختلفة معايير معدلة. توفر ليزر الألياف مزايا مع المواد العاكسة للغاية مثل النحاس أو الذهب.
البوليمرات –؛ يمكن لأشعة الليزر عالية الطاقة أن تذيب بشكل انتقائي مجموعة واسعة من البوليمرات البلاستيكية الحرارية للحام أو التصنيع المضاف. تتطلب بعض البوليمرات مثل PTFE مصادر أشعة متخصصة.
المركبات –؛ يمكن معالجة البوليمرات المقواة بالألياف إلى حد محدود إذا تم التحكم في المعلمات لتجنب التلف الحراري للألياف.
السيراميك –؛ تتطلب المواد الخزفية أجهزة ليزر عالية الطاقة متخصصة للغاية للمعالجة بسبب طبيعتها الحرارية الحرارية الحرارية الحرارية الضعيفة.
السيليكون –؛ يمكن لليزر تقليم وحفر وقطع رقائق السيليكون لتطبيقات الإلكترونيات الدقيقة. ويجري أيضًا استخدام تقطيع البلازما.
الماس –؛ نظرًا لصلابته ومقاومته الكيميائية، لا يمكن تشكيل الماس عمليًا إلا باستخدام الليزر.
يعد اختيار معلمة الحزمة المناسبة والتحكم فيها أمرًا بالغ الأهمية لتحقيق المعالجة السليمة ومنع التلف الحراري غير المنضبط عند العمل مع مواد مختلفة. يعد تطوير المعالجة خطوة أولى مهمة.
ما هي عمليات الشعاع الكهربائي الشائعة؟
هناك العديد من عمليات التصنيع الراسخة التي تستفيد من مصادر طاقة الأشعة الكهربائية:
القطع بالليزر
- يستخدم القطع بالليزر ليزر عالي الطاقة مُركّز لإذابة/تبخير المواد وتحفيز القطع
- يمكن قطع المقاطع الجانبية المعقدة ثنائية الأبعاد من الصفائح المعدنية والألواح والأنابيب بأقل قدر من التشويه
- العملية دقيقة للغاية وقابلة للتكرار من أجل التشغيل الآلي
اللحام بالليزر
- تسمح طاقة الليزر المركزة باللحام الدقيق للمكونات المصغرة أو المواد الرقيقة
- يقلل الإدخال الحراري المنخفض من التشويه وهو أمر ضروري للأجزاء الحساسة
- يمكن لحام المواد غير المتشابهة والمتفاعلة في ضوء التصميم المناسب للمفصل
الحفر/الاستئصال بالليزر
- يمكن لليزر حفر ثقوب في المعادن والبوليمرات والمواد المركبة دون تآكل الأدوات
- يمكن لتقنيات القرع والنقر أن تخلق ثقوبًا بأقطار دقيقة وتشطيبات نهائية
- تتيح المعالجة متعددة النبضات ثقوبًا ذات نسبة عرض إلى ارتفاع عالية جدًا
الوسم بالليزر
- الوسم بالليزر هو طريقة غير تلامسية لتمييز الأجزاء لتحديد الهوية أو العلامة التجارية أو التتبع
- ينشئ علامات سطحية دائمة دون إزالة المواد أو تشويهها
- يمكن تمييز المعادن والبلاستيك والسيراميك والورق والجلد والعديد من المواد الأخرى
الكسوة بالليزر
- تقوم الكسوة بالليزر بترسيب مادة طلاء على جزء باستخدام شعاع ليزر مركز
- يحقق الترابط المعدني حيث يذوب مسحوق الكسوة في الركيزة
- يسمح ببناء طلاءات مقاومة للتآكل أو مقاومة للتآكل
تقشير الصدمات بالليزر
- يستخدم تقشير الصدمات بالليزر نبضات عالية الطاقة لإحداث موجات صدمية تنقل الضغوط الانضغاطية
- يمنع الإجهاد الانضغاطي بدء التشقق الناتج عن الإرهاق ويحسن من عمر الجزء
- يتم إنشاء ضغوط انضغاطية أعمق مقارنةً بالتقشير بالخردق التقليدي
تصنيع المضافات الليزرية
- يُعرف أيضًا باسم الانصهار القاعي للمسحوق بالليزر أو التلبيد المباشر للمعادن بالليزر أو الصهر الانتقائي بالليزر
- عملية الطباعة ثلاثية الأبعاد طبقة بعد طبقة باستخدام المسحوق المعدني والصهر بالليزر
- يسمح بالأشكال الهندسية المعقدة والأجزاء المخصصة مباشرةً من بيانات CAD ثلاثية الأبعاد
اللحام بالشعاع الإلكتروني
- يستخدم شعاع إلكترون مركز لتوفير اختراق عالي للحام في الفراغ
- مثالية لربط المعادن التفاعلية مثل التيتانيوم المعرضة للتلوث الجوي
- يمكن لحام المقاطع السميكة للغاية بسبب القدرة على الاختراق الكامل
التصنيع بالحزمة الإلكترونية المضافة
- يُعرف أيضًا باسم الاندماج القاعي للمسحوق بالحزمة الإلكترونية أو الصهر بالحزمة الإلكترونية
- بناء أجزاء معدنية ثلاثية الأبعاد من المسحوق المعدني باستخدام مصدر حرارة شعاع الإلكترون
- تتجنب العملية التي يتم التحكم فيها بيئيًا مشاكل أكسدة المواد
اللحام بقوس البلازما
- يستخدم اللحام بقوس البلازما نفاثة بلازما ضيقة لتركيز الطاقة الحرارية
- يمكن أن تخترق المقاطع السميكة بالكامل بتمريرة واحدة على عكس اللحام التقليدي
- إنتاج طبقات لحام بأقل قدر من التشوه وخصائص معدنية ممتازة
قطع القوس بالبلازما
- يستخدم نفاثة بلازما عالية الحرارة لإذابة/أكسدة المواد لتحفيز القطع
- لديها قدرة قطع عالية السرعة للصفائح السميكة ويمكنها إنتاج حواف ذات جودة عالية
- تيار بلازما يركز الحرارة في منطقة ضيقة لقطع دقيق
هناك العديد من العمليات المتخصصة الأخرى مثل التقشير بالليزر والتزجيج بالليزر والتلدين بالليزر والحفر بثقب المفتاح بالبلازما وغيرها من العمليات المتخصصة التي تستفيد من طاقة الحزمة بطرق متخصصة. وتستمر القائمة في النمو مع التقدم في تكنولوجيا توليد الأشعة وقدرات التحكم.
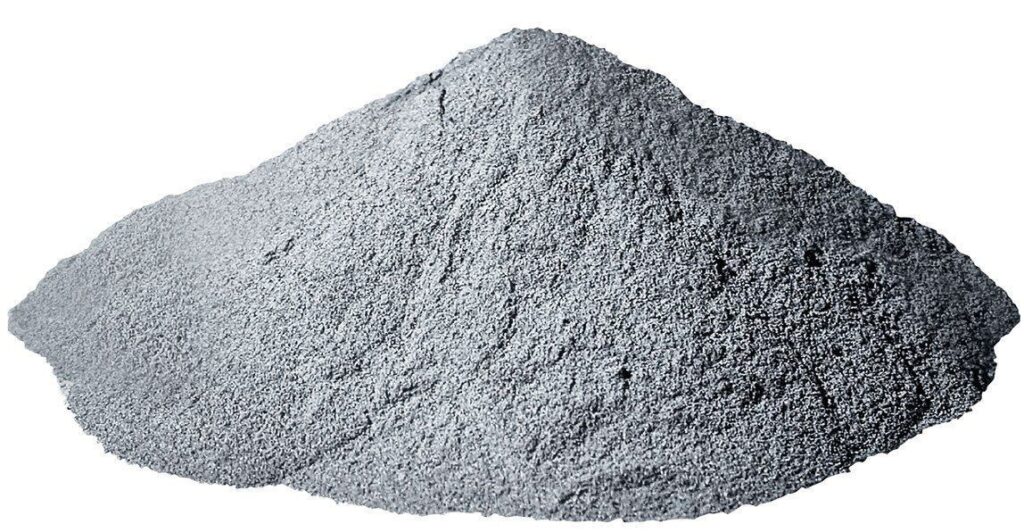
كيف يعمل ضمان الجودة مع هذه العمليات؟
تعتبر ممارسات مراقبة الجودة وضمانها المتسقة مهمة لأي عملية تصنيع. وتشمل بعض جوانب الجودة الرئيسية لعمليات الحزم الكهربائية ما يلي:
- مراقبة العمليات –؛ تحتوي العديد من الماكينات على مستشعرات لمراقبة طاقة الشعاع وموضعه وتركيزه وتدفق الغاز ومستويات التفريغ وما إلى ذلك أثناء المعالجة. يتم تسجيل البيانات وتحليلها.
- الفحص أثناء العملية –؛ يمكن لأنظمة رؤية الماكينة فحص الأجزاء بصريًا أثناء المعالجة بحثًا عن العيوب والمعالجة غير السليمة. وهذا يتيح اتخاذ إجراءات تصحيحية سريعة.
- الفحص اللاحق للعملية –؛ يتم فحص الأجزاء بعد الإنتاج باستخدام القياس البصري، والتصوير بالأشعة السينية، والاختبار بالموجات فوق الصوتية، والاختبار الميكانيكي، وفحص المعادن، إلخ.
- تحسين البارامتر –؛ يساعد تصميم التجارب على تحديد المعلمات المثالية لتلبية المتطلبات. يتم التحقق من صحة المعلمات من خلال عمليات بناء متعددة.
- الصيانة والمعايرة؛ المعايرة –؛ تتطلب مولدات الشعاع وأنظمة تحديد المواقع صيانة دورية وإعادة معايرة لضمان التشغيل المستقر.
- تدريب المشغلين –؛ يضمن التدريب الشامل لمشغلي الماكينات ومهندسي العمليات التشغيل السليم.
- التتبع –؛ تساعد بيانات تحديد الأجزاء وبيانات الإنتاج على تتبع الأجزاء النهائية إلى جميع معلمات العملية وحصص المواد الخام.
- الامتثال للمعايير –؛ يتم تطبيق مبادئ صارمة لإدارة الجودة وفقًا لمعايير المنظمة الدولية لتوحيد المقاييس ISO ومعايير الصناعة.
- التحكم في العمليات –؛ التحكم الإحصائي في العمليات باستخدام مخططات التحكم يتتبع استقرار العملية ويكتشف الانحرافات عن الظروف الاسمية.
مع البنية التحتية المناسبة للجودة، توفر عمليات الشعاع الكهربائي معالجة متسقة للغاية وقابلة للتكرار. يمكن تحقيق التفاوتات الضيقة وخصائص المواد والأداء بسهولة.
ما هي احتياطات السلامة المطلوبة؟
يتطلب تصنيع الشعاع الكهربائي بعض تدابير السلامة الفريدة من نوعها:
- سلامة الليزر –؛ يجب اتخاذ الاحتياطات اللازمة لتجنب التعرض المباشر للعين أو حروق الجلد. يجب ارتداء النظارات الواقية والحواجز والجلود وأجهزة الإنذار والأجهزة البينية.
- مخاطر شعاع الإلكترون –؛ يتم توليد أشعة سينية شاردة تتطلب التدريع. كما تتطلب أشعة الإلكترونات الشاردة الاحتواء.
- استخلاص الدخان –؛ تولد العديد من عمليات الأشعة أبخرة اللحام أو الجسيمات أو الغازات الخطرة التي تتطلب استخلاص أبخرة موضعية.
- المخاطر الكهربائية –؛ تنطوي مولدات الشعاع على جهد عالٍ يستلزم عزلًا وفصلًا وتداخلات وصول مناسبة.
- مخاطر الحرائق –؛ الأبخرة والزيوت والأتربة القابلة للاشتعال تخلق خطر نشوب حريق مع طاقة الشعاع. التدبير المنزلي السليم أمر بالغ الأهمية.
- التعرض للضوضاء –؛ تنتج بعض عمليات الشعاع مثل القطع بقوس البلازما ضوضاء عالية تستلزم حماية السمع.
- المخاطر المريحة –؛ يمكن أن يؤدي الرفع غير السليم والانحناء والحركات المتكررة إلى إجهاد أو إصابات أخرى.
يمكن إدارة هذه المخاطر بفعالية من خلال الضوابط الهندسية المناسبة، والسياسات الإدارية، ومعدات الحماية الشخصية (PPE)، والتدريب، من أجل مكان عمل آمن. كما أن التصميم الدقيق للمرافق والمعدات ضروري أيضًا لعزل مخاطر الأشعة.
كيف يتم تصميم الأجزاء ومحاكاتها لعمليات الشعاع؟
تساعد العديد من الأدوات البرمجية في تصميم ومحاكاة الأجزاء الخاصة بتصنيع الحزمة الكهربائية:
- برنامج CAD –؛ تسمح برامج التصميم بمساعدة الحاسوب بنمذجة تفصيلية ثلاثية الأبعاد لهندسة المكونات المحسّنة للتصنيع الإضافي. يمكن دمج قيود التصميم.
- تحسين الطوبولوجيا –؛ يمكن للخوارزميات تحسين تخطيط المواد لتحسين الأداء تحت التحميل. يتيح ذلك إمكانية تخفيف الوزن بشكل كبير.
- برامج المحاكاة –؛ يحاكي تحليل العناصر المحدودة وديناميكيات الموائع الحسابية الضغوط ودرجات الحرارة وتأثيرات التشويه والتفاعلات الحرارية الهيكلية والضغوط المتبقية وما إلى ذلك. يتيح ذلك التحقق من صحة التصميم.
- نمذجة العمليات –؛ يمكن للبرامج المتخصصة محاكاة تفاعل شعاع الطاقة مع طبقات المسحوق لنمذجة تجمعات الذوبان وتدرجات الحرارة والتبخير وما إلى ذلك. وهذا يضمن جدوى العملية.
- تخطيط البناء –؛ تقوم معالجات البناء بتقطيع نماذج التصميم بمساعدة الحاسوب وإنشاء مسارات أدوات مثالية لأنظمة الإضافات التي تأخذ في الحسبان الدعامات وتراكم الحرارة والضغوط المتبقية وغيرها.
- تخطيط التفتيش –؛ يمكن تحديد متطلبات الفحص في نماذج CAD. ثم تقود النماذج إجراءات الفحص الآلي.
- بيانات الماكينة –؛ يتم دمج بيانات الماكينة الحقيقية في عمليات المحاكاة لمزيد من الدقة. يضمن التحقق من صحة الحلقة المغلقة إمكانية تصنيع التصميمات.
تمكّن القوة المتزايدة لعمليات المحاكاة المقترنة بالتحقق من صحة البناء الحقيقي من "التصميم من أجل قابلية التصنيع". وهذا يقلل من وقت التطوير والتكاليف مع ضمان الجودة العالية.
كيف تتم أتمتة الأنظمة والتحكم فيها؟
تستفيد عمليات الشعاع الكهربائي الحديثة من الأتمتة والتحكم الشاملين:
- التحكم باستخدام الحاسب الآلي الرقمي –؛ تضع أنظمة التحكم الرقمي باستخدام الحاسب الآلي متعددة المحاور الحزمة و/أو قطعة العمل بدقة عالية من خلال مسارات معقدة.
- التحكم في الحركة –؛ توفر المحامل الكهرومغناطيسية الدقيقة والمحركات الخطية والترميز البصري حركة سلسة ودقيقة.
- معالجة عالية السرعة –؛ تقوم أجهزة الجلفانومتر والمرايا متعددة الأضلاع وغيرها من التقنيات بمسح الطاقة وتوزيعها بسرعة للحصول على معدلات معالجة عالية.
- تتبع التماس –؛ تتيح المراقبة من خلال العدسة والمراقبة القائمة على الكاميرا إمكانية التحكم التكيّفي لمتابعة طبقات اللحام أو المعالجة الصحيحة في الوقت الفعلي.
- معالجة الشعاع المتقدم التركيز الديناميكي، وتقسيم/تشكيل الشعاع، والبصريات المتخصصة التي تتحكم بدقة في توزيع طاقة الشعاع.
- رؤية الآلة –؛ تضمن المراقبة البصرية أثناء العملية لهندسة حوض الذوبان والانبعاثات الحرارية والهندسة ضمان الجودة. يتم تمكين التحكم في الحلقة المغلقة.
- المناولة الدقيقة للمساحيق –؛ يتيح ترسيب المسحوق وتنعيمه وإعادة طلائه الذي يتم التحكم فيه بعناية تصنيع إضافات عالية الدقة.
- الغلاف الجوي الخامل –؛ تمنع أنظمة التفريغ أو غازات التغليف الخاملة أكسدة المواد.
- القياس أثناء المعالجة –؛ توفر المراقبة الموقعية لدرجات الحرارة والانبعاثات وبيانات قياس الأبعاد ملاحظات حية عن العملية.
- المعالجة اللاحقة المتكاملة –؛ يمكن دمج خطوات مثل المعالجة الحرارية والتشغيل الآلي باستخدام الحاسب الآلي والتشطيب السطحي والفحص للحصول على خلية تصنيع مؤتمتة كاملة.
تتيح هذه التقنيات إنتاجًا غير مأهولاً بدون طيار ومعالجة سريعة واتساقًا عبر ملايين دورات الإنتاج. كما يتم تمكين التحسين المستمر من خلال جمع البيانات والتحليلات.
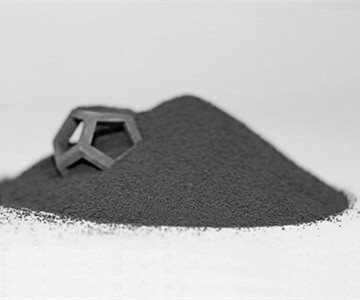
ما هي بعض الأمثلة على التطبيقات والصناعات؟
يشمل تصنيع العوارض الكهربائية العديد من الصناعات والمنتجات:
الفضاء –؛ التصنيع الإضافي للهياكل المعقدة المصنوعة من التيتانيوم وسبائك النيكل، والحفر الدقيق واللحام للمحركات.
الطبية –؛ التصنيع الإضافي للغرسات الخاصة بالمريض، وقطع الدعامات والأدوات الجراحية بالليزر.
السيارات –؛ لحام الفراغات المصممة خصيصًا، وقطع أنماط الوسائد الهوائية، وحفر فوهات حاقن الوقود.
الإلكترونيات –؛ تقطيع الرقاقات والإلكترونيات بالليزر وحفرها ووضع العلامات عليها. لحام الوصلات البينية.
التكنولوجيا الدقيقة –؛ التصنيع الإضافي أو التصنيع الآلي للمكونات متناهية الصغر بتفاصيل أقل من 100 ميكرون.
الأدوات والقوالب –؛ القطع بالليزر لأدوات القوالب. النماذج الأولية السريعة للأدوات.
المجوهرات والساعات –؛ الحفر والقطع بدقة متناهية. وسم الأرقام التسلسلية الفردية بالليزر.
الأبحاث –؛ تستخدم الجامعات التصنيع الإضافي بالليزر في الهياكل الشبكية الدقيقة وأبحاث التحكم ودراسات المعادن وغيرها.
يستمر نطاق التطبيقات التجارية والبحثية في النمو بسرعة بفضل مرونة ومزايا التصنيع بالأشعة الكهربائية.
ما الذي يحمله المستقبل لعمليات الشعاع الكهربائي؟
تعمل العديد من التطورات المثيرة على توسيع قدرات الشعاع الكهربائي:
- الأنظمة متعددة الأشعة وأنظمة الليزر المتعددة –؛ ستؤدي الاستفادة من الحزم المتعددة إلى زيادة معدلات البناء وتعزيز التحكم.
- الليزر فائق السرعة –؛ توفر ليزر بيكو ثانية وليزر الفيمتو ثانية إمكانيات جديدة لمعالجة المواد مع الحد الأدنى من المناطق المتأثرة بالحرارة.
- ليزر عالي الطاقة –؛ تستمر قوى الليزر في التوسع إلى عشرات الكيلوواط مما يتيح معدلات بناء أسرع ومقاطع أكثر سمكًا ومكونات أكبر. كما تعمل تصاميم الليزر الجديدة على تحسين كفاءة التوصيل بالجدار.
- التصنيع الهجين –؛ يوفر الجمع بين الترسيب بالليزر مع التصنيع الآلي باستخدام الحاسب الآلي أو عمليات أخرى في ماكينة واحدة إمكانات جديدة.
- مواد جديدة –؛ يتم تطوير سبائك الألومنيوم الجديدة وسبائك الألومنيوم عالية الانتروبيا ومركبات المصفوفة المعدنية وغيرها من المواد للاستفادة من خصائص التصنيع الإضافي.
- القياس أثناء المعالجة –؛ سيتيح التقدم في الاستشعار عالي السرعة والتحكم في الحلقة المغلقة إمكانية التصحيح في الوقت الحقيقي والتحسين الذاتي أثناء عمليات البناء.
- المحاكاة –؛ مع استمرار تحسن دقة المحاكاة، ستصبح النمذجة والتحقق من الصحة أسرع وأكثر موثوقية.
- تكامل النظام –؛ سيؤدي التكامل الأكثر إحكامًا بين أنظمة المسح الضوئي ومناولة المسحوق والمعالجة اللاحقة للمعالجة والأتمتة ومراقبة الجودة إلى تحسين المتانة.
يستعد تصنيع الأشعة الكهربائية للنمو المستمر وسيقود الابتكارات في العديد من قطاعات التكنولوجيا. ستتم الاستفادة من القدرات الفريدة لعمليات الليزر وأشعة الإلكترون والبلازما بطرق جديدة لم يتم تصورها بعد. أوقات مثيرة في المستقبل!
التعليمات
فيما يلي بعض الأسئلة الشائعة الشائعة حول عمليات تصنيع الشعاع الكهربائي:
ما هي أنواع الليزر المستخدمة في التصنيع؟
تشمل أشعة الليزر الصناعية الشائعة ليزر الألياف وليزر ثاني أكسيد الكربون وليزر الصمام الثنائي وليزر الصمام الثنائي وليزر الأقراص وليزر الصمام الثنائي المباشر. يقدم كل منها مزايا مختلفة من حيث مستويات الطاقة وجودة الحزمة والأطوال الموجية والتكلفة. من المهم مطابقة نوع الليزر مع المادة والتطبيق.
كيف يمكن مقارنة التصنيع المضاف بالليزر بالطرق الأخرى؟
يوفر التصنيع باستخدام الليزر AM دقة عالية جدًا وخصائص مواد ممتازة. ومع ذلك، يمكن لعمليات التصنيع باستخدام شعاع الإلكترون AM البناء بشكل أسرع قليلاً وبتكاليف أقل. تتميز عملية التصنيع باستخدام النفاثات النفاثة الموثقة بأقل التكاليف ولكن بخصائص مواد أضعف. يعتمد الاختيار الصحيح على التطبيق.
ما أنواع أنظمة الأشعة الإلكترونية المستخدمة؟
تولد أنظمة التفريغ العالي المزودة بفتائل التأين الحراري أو كاثودات التنجستن حزمًا ثابتة ومستمرة. توفر أنظمة التفريغ المنخفض مع كاثودات البلازما حزمًا نابضة. تتراوح طاقة الحزمة من بضعة كيلووات فقط إلى حوالي 100 كيلووات للتصنيع الإضافي.
كيف يعمل الوسم بالليزر؟
يزيل الوسم بالليزر الطلاء السطحي، أو يلدّن المادة الأساسية، أو يُحدث تغييراً في اللون من خلال الأكسدة أو الكربودة أو التغيرات الكيميائية في السطح. يتم اختيار أطوال موجية مختلفة بناءً على المادة.
ما مدى سماكة القطع أو اللحام بالليزر والبلازما؟
يمكن لأجهزة الليزر الليفي أن تقطع الفولاذ بشكل موثوق حتى سمك بوصة واحدة تقريبًا. يمكن للقطع بالبلازما قطع أكثر سمكًا بكثير –؛ حتى حوالي 6 بوصات للفولاذ الطري. يقتصر عمق اللحام بالليزر على حوالي 0.25 بوصة للاختراق الكامل. يمكن أن يحقق اللحام بثقب المفتاح بالبلازما لحامًا كامل الاختراق بتمريرة واحدة على الفولاذ بسُمك 6-8 بوصات.
ما أنواع العيوب التي يمكن أن تحدث في اللحام بالليزر؟
تشمل العيوب الشائعة في اللحامات بالليزر المسامية الناتجة عن انحباس الغازات والتشقق الناتج عن انكماش التصلب والانصهار غير الكامل والتقويض والتشويه والتناثر. هناك حاجة إلى التصميم المناسب للمفاصل والتركيبات والمعايير والتحكم السليم.
ما هي احتياطات السلامة المطلوبة في اللحام بالشعاع الإلكتروني؟
وتتطلب الأشعة السينية الفارادية المتولدة تدريعاً مناسباً. كما يجب احتواء شعاع الجهد العالي بشكل صحيح. ويحتاج المشغلون وموظفو الصيانة إلى تدريب متخصص للعمل بأمان مع أنظمة الأشعة الإلكترونية.
كيف يمكن المقارنة بين ثقب الصدمات بالليزر وثقب الصدمات بالليزر؟
يضفي الصقل بالصدمات بالليزر إجهادات انضغاطية تصل إلى 6 أعمق من الصقل بالخردق. كما أنه يتجنب خشونة السطح.